Fluorescence is a process by which molecules absorb photons of one color band (typically lower wavelengths) and emit photons at another color (typically higher wavelengths). Filters are used to separate these colors from one another. Below we describe the underlying processes of the fluorescence spectrum and the origin of the fluorescence lifetime.
In order to understand fluorescence spectra, it helps to have an idea of what is going on in the molecules when they interact with light. In the diagram on the left below (a Jablonski diagram), the groups of lines labelled So and S1 represent electronic energy levels (states) of a molecule. There can be many of these at higher and higher energy. The lowest energy is at the bottom of the Jablonski diagram and the length of the arrows is proportional to the energy of the transition. Energy is inversely proportional to wavelength, so the longer the arrow, the shorter the wavelength in the spectrum. Within each energy level are a number of vibrational levels (labelled v = 1-4) and rotational levels (unlabelled).
Absorption or excitation-
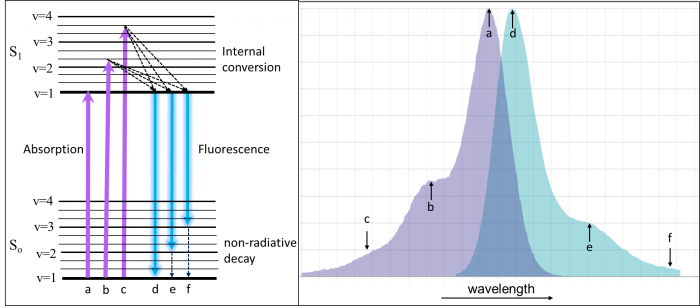
Molecules spend most of their lives in the ground state (So). When a photon with the correct energy interacts with the molecule, it is absorbed (purple lines) and ends up in the excited state (S1). Because there are many molecules that have slightly different solution environments and energy levels, the absorption spectrum is broadened into the spectrum that we typically see (the purple area on the left). Often, it is still possible to make out the vibrational levels of the excited state (labelled a-c). In a gas-phase absorption spectrum, each vibrational and rotational line is exquisitely visible. Using high-powered pulsed laser systems, multiphoton (MP- usually 2 or 3 photons) absorption can be achieved. In this case, multiple low-energy (typically red or IR) photons add up to equal the energy of the purple transitions. The photons must reach the sample within 1 fs (10-15 seconds) of one another to achieve MP absorption.
Fluorescence and the Stokes shift-
Once in the excited state, the molecules relax via internal conversion to the lowest vibrational level in S1 (within a few nanoseconds). This relaxation is often the origin of the “Stokes shift”. Stokes shift becomes important when choosing fluorescence filters and when designing fluorescence experiments with many simultaneous labels. From v=1 of S1, fluorescence occurs (teal glowing arrows); photons are emitted and the molecule finds itself back in the ground state (So). Similar to absorbance, the ground state vibrational levels can often be seen in the fluorescence spectrum as well (d-f). The fluorescence is always at a lower energy (longer wavelength) than the absorption because of internal conversion and non-radiative decay processes. The notable exception is when the absorption is performed with multiphoton processes. In that case, the fluorescence is always at a higher energy (lower wavelength) than the absorbed photons.
Other excited state relaxation processes-
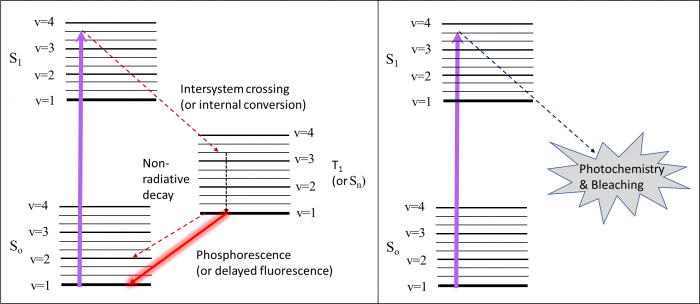
Frustratingly, fluorescence is not the only thing that can happen after a molecule enters the excited state. Sometimes there are lower energy excited states that are not directly accessible from absorption. These include states that are “not allowed” quantum mechanically, as in a singlet-triplet transition (T1 in the diagram), or other singlet states that aren’t allowed because of symmetry or other selection rules (Sn in the diagram). From S1, the molecule can (1) undergo intersystem crossing to T1 followed by phosphorescence or non-radiative decay or (2) internal conversion to Sn which can result in delayed fluorescence or non-radiative decay back to the ground state. Sometimes molecules can become trapped in these “dark states” for some time. One good of example of this is the “blinking” that occurs in quantum dots.
Sometimes the excited state, which tends to be more reactive, undergos a chemical reaction. This can include oxidation, isomerization, degradation or other mechanisms. While useful in the case of "caged" molecules (where a moiety is released after illumination), this is also the root of photobleaching. While photobleaching can be useful in some experiments (FRAP – fluorescence recovery after photobleaching), it is generally an undesirable result.
Fluorescence lifetime-
All of these processes compete for the excited state. Each molecule takes a single path back to the ground state. Adding up the emission from all the excited molecules (or repeated measurements on the same molecule) leads to the reported fluorescence lifetime. In other words, the fluorescence lifetime is a statistical phenomenon. The longer the fluorescence lifetime, the higher the fluorescence quantum yield; fluorescence is the most likely outcome of an absorption event. As the likelihood of other processes increase, the fluorescence lifetime decreases as well as the quantum yield.